Thinking about stars as living beings is not entirely unreasonable. After all, they are born, grow, die, and even reproduce, just like living organisms do, even if they go through these life stages in a slightly different order. Interestingly, they reproduce after death. Supernovas–those tremendous explosions that mark a turning point in the active life of some stars, which can outshine the entire galaxy that contains them–can give rise to new celestial bodies, and even new stellar systems.
Delving into the life of a star in a handful of paragraphs is obviously impossible. This fascinating topic can give writers fodder for dozens of books. Our aim here is to explain, in the most didactic and accessible way possible, the stages a star goes through from the moment it begins to form under the inexhaustible influence of gravity until it reaches the final stages of its active life and collapses, potentially transforming into a neutron star, a quark star, or even a black hole. Having said that, let’s embark on our journey.
A Star is Born
Every star is unique, and each has its own “personality.” However, the mechanism that triggers their birth is always the same. In a way, we can consider them all to be related. Stars are born from clouds of dust and gas that are scattered throughout the universe; these began to form shortly after the Big Bang, nearly 14 billion years ago, according to the estimates of some scientists.
Research conducted by several different groups suggests that the first stars appeared shortly after the universe was formed. In fact, it is believed that the oldest known star was born a staggering 13.6 billion years ago, making it almost as old as the universe itself. The team of astronomers from Australian National University that was responsible for its discovery states that it is sixty times larger than our Sun and is located in our own galaxy, the Milky Way, 6,000 light-years away from Earth. Practically next door.
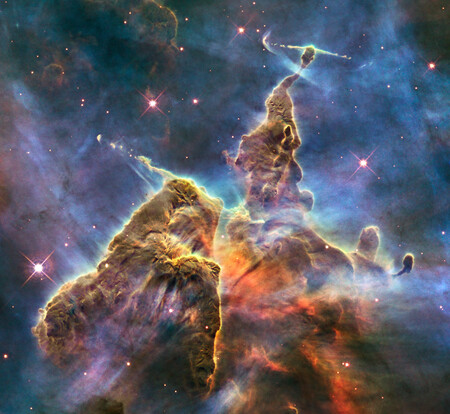
However, astronomers are convinced that there are even older stars out there. This hypothesis is supported by the fact that our 13.6-billion-year-old giant consists not only of hydrogen but also of carbon, magnesium, and calcium, elements that had to have been previously produced by one or more stars from an even older generation, with very low metallicity. In this case, we consider “metals” to be all chemical elements that are heavier than helium, regardless of their position in the periodic table.
To start out on our journey, we must look into the composition of stars. This is fundamental, as it greatly influences their evolution. A star’s life is intimately linked not only to its initial composition, but also, above all, to its mass, which is simply the amount of matter that gravity can gather and condense into a portion of space. The force of nature responsible for a stars’ birth is known as gravitational contraction, an inexhaustible phenomenon that gradually gathers and compacts the elements that later on, under specific conditions we’ll delve into shortly, will produce a new star.
As we mentioned earlier, all stars are different, because their mass and initial chemical composition are also different. Still, we can assume that approximately 70% of their mass is hydrogen (protium, technically, an isotope of hydrogen with a single proton in its nucleus and an orbiting electron); between 24 and 26% is helium, and the remaining 4 to 6% is a combination of elements heavier than helium, to which astrophysicists commonly refer to as metals. As you can see, the proportion of metals in a stars’ total mass is low compared to the amount of helium and, especially, hydrogen that they contain—but it is of utmost importance, as these elements, which can vary greatly from one star to another, have a crucial impact on their evolution.
It’s also worth noting that any minimal variation that occurs around that initial 70% of hydrogen will directly impact the life of each star. In any case, as we’ve seen, the true engine capable of birthing a new star is gravity. This force is responsible for gathering and compressing these elements, gradually heating them. If the amount of matter accumulated through gravitational contraction is large enough and the temperature reached high enough, then the “nuclear furnace” will ignite.
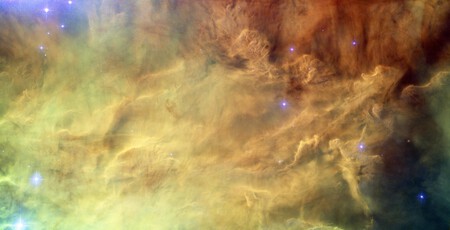
The core of this celestial body, which is the part with a higher pressure and temperature, is where the hydrogen nuclei will begin to fuse and form new helium nuclei, releasing huge amounts of energy in the process. The moment the nuclear furnace ignites and fusion reactions between hydrogen nuclei begin is when we we can state that a new star has been born.
This natural process is what we replicate in the experimental nuclear fusion reactors we’ve built in the past, as well as those we’re currently building, such as the International Thermonuclear Experimental Reactor (ITER). The difference is that the hydrogen isotopes we use are deuterium and tritium, not protium, as the former require slightly more attainable conditions of pressure and temperature and, therefore, are easier to achieve.
At this point, and before moving forward, it’s important to stop for a moment to find out how much matter needs to be accumulated through gravitational contraction to ignite the nuclear furnace that will give rise to the birth of a star. During the formation of the protostar, which is the object whose “oven” has not yet ignited, gravity continues to incorporate matter from the molecular gas cloud of the interstellar medium I mentioned at the beginning of the article, causing the core to compress and heat up further.
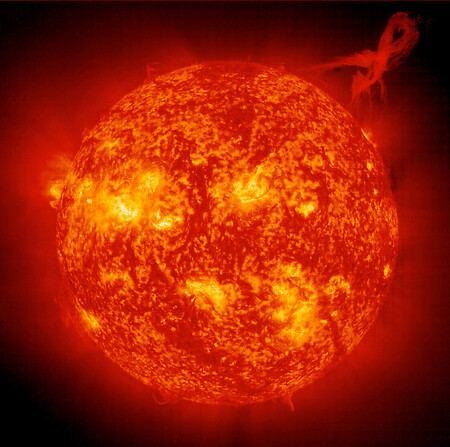
The ignition of hydrogen through nuclear fusion processes occurs when the core temperature of the protostar reaches 10 million degrees Celsius, initiating a process astronomers refer to as the main sequence, which will last throughout most of the star’s life. But there’s another possibility that we must consider. If the object in formation has less than 0.08 solar masses, core compression and heating will cease before reaching the temperature necessary to initiate the fusion of the hydrogen nuclei. It will gradually cool down, and several million years later it will turn into a brown dwarf.
A simple way to understand a brown dwarf is thinking about it as a celestial body that aspired to become a star but ultimately failed because it couldn’t gather the necessary mass. Still, astrophysicists believe that, depending on the amount of matter that the gravitational contraction managed to condense, some brown dwarfs can fuse deuterium, lithium, and tritium, as those elements are easier to “burn” than protium, which, as we saw earlier, is common hydrogen, the one with no neutron in its nucleus.
However, this activity usually doesn’t last long; typically, it extends only through their youth. The fact that they fail to sustain nuclear fusion reactions from protium nuclei causes them to gradually contract and cool down until they reach equilibrium. When the residual heat from fusion reactions–if there are any–dissipates, they stop shining. The celestial bodies they end up becoming are something between low-mass stars and giant gas planets like Jupiter.
After Ignition, Stars Reach Their Prime
A star’s longevity is closely linked to its mass. The more massive ones consume their fuel–hydrogen–more quickly, through the nuclear fusion process we discussed earlier, depleting their energy sources in less time. Consequently, they have a shorter active life. This is the main sequence stage, as mentioned earlier. The combustion of hydrogen in the core causes this element to gradually deplete, forcing the star to readjust by contracting the core to increase its temperature and stop the gravitational collapse it would undergo if it wasn’t able to reach equilibrium through radiation pressure and gas pressure.
The equilibrium found by a star during the hydrogen-burning phase, which is the longest period of its life, is possible because gravitational contraction. This pulls matter toward its interior and is balanced by the gas and radiation pressure emitted by the star, which push matter outward.
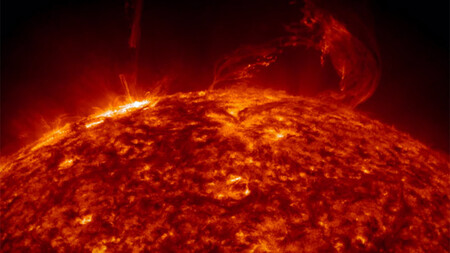
This is a very complex process that constantly requires the star to readjust. Fortunately, we have the help of four differential equations, which, based on a star’s initial chemical composition and mass (and with the aid of powerful computers) allow us to accurately predict its evolution, as well as the moment where gravitational collapse–which we’ll discuss shortly–will take place.
Fear not—we won’t delve into the mathematical complexity of these four equations. Still, it would be useful to briefly describe the information that each of them provides so that we can understand why they help us predict a star’s evolution. But before going over them, one more note: Despite their complexity, all of them come from basic physics. Quantum and relativistic effects, which will be important later when we see how a star’s mass influences what it will transform into when it exhausts its energy sources, are not yet necessary.
The first equation is the mass equation, which assumes that the mass at a star’s center is zero, and in its atmosphere we have the total mass. The second is the hydrostatic equilibrium equation, which reveals what we mentioned earlier: how a star’s gravity counteracts the gas and radiation pressure to maintain the star in equilibrium. The third is the energy production equation, which determines how a star obtains energy from the fusion reactions that take place inside it, as well as through gravitational contraction. The last one is the energy transport equation, which shows how energy is transported from a star’s core outward.
When the temperature and pressure in the star’s core are high enough, as we saw earlier, hydrogen nuclear fusion reactions begin. Now, something we haven’t analyzed yet is the crucial role kinetic energy plays in this phenomenon. It is precisely the increase in temperature that gives hydrogen nuclei the kinetic energy necessary to reach a high enough speed, helping them overcome the natural electric repulsion caused by their positive charge when several of them get close.
Under these conditions, we can picture a star’s core as a sort of extremely dense soup composed mainly of hydrogen nuclei hot enough to be moving and shaking in all directions. Their speed is so high that when they get close enough, their inertia counteracts their natural electric repulsion, and the strong nuclear force, which keeps atomic nucleus particles together, springs into action. From here, fusion between these nuclei is an easy matter.
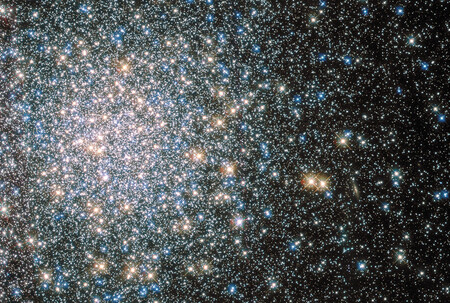
The first chemical element “manufactured” in large quantities in a star’s core from the fusion of hydrogen nuclei is helium. This process entails the release of a large amount of energy. But helium is not the only by-product of nuclear fusion reactions—not by a long shot. As hydrogen is consumed, the star readjusts, compressing its core and increasing its temperature, so that the necessary conditions for helium ignition begin... or not. It all depends on the star’s mass.
If the core is massive enough, it will heat up and compress enough for helium nuclear fusion to take place when hydrogen is depleted. The triple alpha process–which is how the fusion of three helium nuclei to produce a carbon nucleus is known–takes place at temperatures above 100 million Kelvin degrees, which clearly shows the extreme temperature that the star’s core must reach in order for carbon production to occur. And, again, the star continues to readjust so that our four equations continue to yield results consistent with a body in perfect equilibrium.
This in mind, what happens to the carbon in our massive star? Plainly and simply, the same thing that happens with helium. If the star’s mass is big enough, when the carbon in the central core is depleted, it will contract again and increase its temperature to continue the fusion processes, resulting in heavier and heavier elements. Once we reach this stage, it’s easy to understand how the star acquires a structure of concentric layers similar to that of an onion, with a fusion reaction taking place in each of the layers, producing increasingly heavier ashes–understanding that the ashes are made up of the elements that result from the combustion of lighter elements.
As the nuclear fuel burns, the star shifts in the luminosity and temperature diagram; at the same time, its radius and temperature increase. However, these continuous nuclear fusion processes only take place if the star’s mass is very large. If the star is not massive enough, the core temperature will not be hot enough to initiate helium combustion, and it will continue to readjust so that the outer layers are hot enough to allow the remaining hydrogen to burn. This process causes it to expand and acquire a reddish color due to the cooling of its surface. When stars are in this phase of their evolution they are known as red giants.
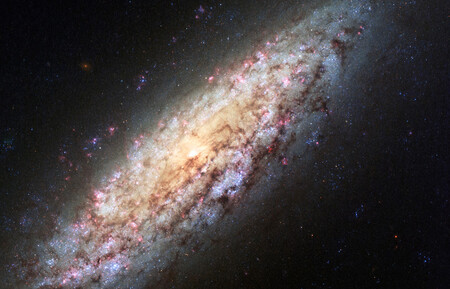
This beautiful image was also taken by the Hubble Space Telescope. What we see here is NGC 6503, a solitary galaxy located 18 million light-years away from us, in the Draco constellation.
Finally, when it completely consumes its fuel, it expels the outermost layers, giving rise to a gas cloud known as a planetary nebula, which usually takes the form of a ring or a bubble. The center of the nebula contains what is left of the star: a degenerate star, also known as a white dwarf.
This celestial body receives its name because it is relatively small; at least much smaller than it was during the main sequence and red giant stages, and initially its temperature is still very high. However, it’s no longer producing energy because its fuel has run out, so it gradually cools down until it stops emitting any detectable radiation. At that point, they become known as black dwarfs, because the absence of radiation prevents them from being detected. On a very curious note, astrophysicists are convinced that there are no black dwarfs in the universe yet, because white dwarfs take such a long time to cool down that not even the oldest ones have stopped emitting radiation.
Our Sun is a star with relatively little mass, so it will end its days expanding and transforming into a red giant before expelling its outermost layers into the stellar medium and remaining in space in the form of a white dwarf. But rest assured: Current mathematical models show that it has only consumed approximately 50% of its fuel, and it has an approximate age of about 4.6 billion years. It won’t run out of hydrogen for almost another five billion years, which will be the moment when its main sequence will come to an end.
Neutron Stars, Quark Stars, Black Holes
We already know how low-mass stars end their days, but we still need to find out what happens to high-mass stars. Up to this point, I’ve established the difference in a somewhat ambiguous way, but the time has come to connect the dots.
Thanks to the Indian astrophysicist Subrahmanyan Chandrasekhar, we know with considerable precision what the mass limit of a star is so that, instead of ending its days as a white dwarf, it transforms into a neutron star. The Chandrasekhar limit, as this value is known, is equal to 1.44 solar masses. This simply means that if the mass of a white dwarf exceeds that limit–taking the mass of our Sun as a reference–it will not end its days as a white dwarf, but will collapse into a neutron star, instead. But we know even more: We also know the mass limit that neutron stars can bear, in this case thanks to the research of Richard Chace Tolman, Julius Robert Oppenheimer, and George Michael Volkoff.
The original Tolman-Oppenheimer-Volkoff limit was proposed in 1939, but it has been corrected in subsequent decades with new findings by astrophysicists, and with the help of new measuring instruments. Today, scientists believe it to be approximately 2.17 solar masses. This means–as we saw when we talked about the Chandrasekhar limit applied to white dwarfs–that if a neutron star exceeds this value, it will collapse to become a quark star or a black hole. Quark stars have not yet been conclusively observed, but astrophysicists are currently studying several neutron stars that could in fact be quark stars.
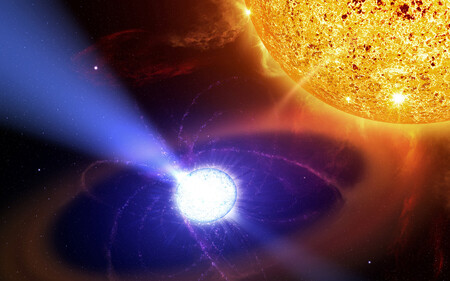
This recreation represents AE Aquarii, a binary system consisting of two stars: a white dwarf and an orange star. Interestingly, the white dwarf emits X-ray pulses as it rotates on its axis, which makes it similar to pulsars.
Now, before moving forward, let’s take a look back for a moment. As we’ve seen, the most massive stars progressively burn their hydrogen, then helium, carbon, and so on, producing increasingly heavier elements inside them. The lighter elements are “manufactured” in the outermost layers, and the heavier ones in the inner layers. But if the star is massive enough, there will come a time when the inner core–the deepest layer of the star–will be made up of iron. Something very interesting happens with this element: No more energy can be extracted from it through nuclear fusion.
When energy production stops in the core of the star, radiation pressure (which tries to expand the star) is unable to counteract gravitational contraction (which tries to compress the star), so the iron core is forced to bear the weight of all the layers of the star above it. That pressure is enormous, and, since the star has lost its balance, the core suddenly contracts, causing all the other layers of material to fall abruptly onto it before they bounce back with extreme violence and are ejected into the stellar medium at very high speeds. This is a supernova. The energy released in these huge explosions is such, that for a few seconds they shine brighter than the entire galaxy they inhabit.
Supernovae are unquestionably one of the most striking cosmic events that we know of. However, what makes them really interesting is their ability to “sow” the interstellar medium with the chemical elements produced by the star through nuclear fusion processes. These elements can later contribute to the formation of new stars and planets, so it’s only reasonable to regard supernovae, which mark the moment when massive stars end their active lives, as the resource they use to reproduce an instant after their last stellar heartbeat.
But that’s not all. The immense pressure to that the iron core of massive stars is subjected to causes very important changes in the structure of matter, which is no longer composed of electrons, protons, and neutrons, like ordinary matter, but is only made up of neutrons. For this reason, neutron stars are nothing more than what’s left when a massive star ends its active stage as a supernova. They are sort of a huge crystal that’s formed only by neutrons.
When I mentioned the four equations that allow astrophysicists to predict how a star’s evolution will take place, I said that they come from basic physics. However, we have also entered the realm of quantum mechanics. In fact, to accurately calculate the famous limit bearing his name, Chandrasekhar was forced to consider quantum and relativistic effects in his calculations.
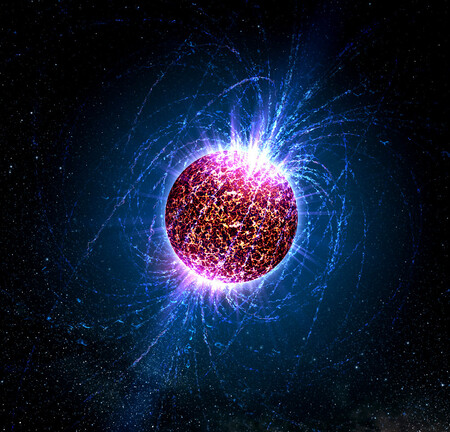
Without quantum physics, our current knowledge of the lives of stars wouldn’t be possible. We also wouldn’t be able to understand the structure of the matter of white dwarfs and neutron stars. And, of course, we wouldn’t be able to even guess what happens inside black holes, something that we still know very little about, but whose mysteries astrophysicists are gradually delving into, largely thanks to the tools provided by quantum physics.
Because of this discipline, astrophysicists have managed to calculate the density of those exotic, exciting objects like white dwarfs and neutron stars with great precision. The former have, no more and no less, an approximate density of one ton per cubic centimeter; in other words, a piece of white dwarf the size of a die weighs roughly about a ton.
Neutron stars are even more impressive. In them, the iron and helium in the core have disintegrated due to the action of photons, very energetic particles that can break down these elements into alpha particles–which are nuclei that have no electrons and, therefore, have a positive electrical charge–and neutrons. Through a mechanism known as beta capture (whose complexity we won’t delve into to avoid complicating this article further), protons are transformed into neutrons. So, as we saw before, a neutron star is only made up of neutrons, and its matter is in a different state than that of the “regular” matter we are familiar with.
What we’ve just discovered helps us to understand what is undoubtedly the most spectacular characteristic of neutron stars: their density. Just a fragment of one cubic centimeter in size weighs about a billion tons. Yes, you read it right. A piece the size of a sugar lump–a billion tons. But that’s not all. As we saw a few paragraphs above, if the mass of the neutron star exceeds the Tolman-Oppenheimer-Volkoff limit, after gravitational collapse we’ll get a quark star, which has an even higher density. Or even a black hole... but that’s a story for another time.
Images | NASA Goddard Space Flight Center
View 0 comments